Decoding the Bandgap: A Comparative Analysis of Energy Levels in Common Semiconductor Materials
Semiconductors have long been the cornerstone of modern electronics. Their properties, especially the ‘band gap’ energy, dictate their performance and utility. This article explores the bandgap energies of common semiconductor materials, offering insight into how these differences impact their applications.
Understanding the Bandgap
The bandgap refers to the energy difference between the valence band and the conduction band in semiconductor materials. It represents the minimum energy required to move an electron from the valence band to the conduction band. It’s a crucial parameter that dictates the intrinsic semiconductor properties and its electronic applications.
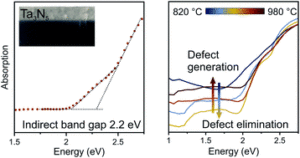
Wide Band Gap Semiconductors: The Powerhouses
Wide bandgap semiconductors (WBGs) are materials with a large band gap. They can operate at higher temperatures, voltages, and frequencies, making them ideal for power semiconductor devices. Popular WBG materials include Silicon Carbide (SiC) and Gallium Nitride (GaN), boasting bandgap energies of 3.26 eV and 3.4 eV, respectively.
Silicon: The Ubiquitous Semiconductor
Silicon (Si) is perhaps the most common semiconductor, integral to a wide range of applications. It has an indirect band gap of 1.12 eV at room temperature. While its relatively small bandgap energy limits its efficiency in some high-power applications, its abundance and ease of fabrication make it the industry standard.
Direct Bandgap Semiconductors: The Light Emitters
Semiconductors with a direct bandgap, such as Gallium Arsenide (GaAs) and Indium Phosphide (InP), can efficiently convert electrical energy into light, making them suitable for solar cells and semiconductor lasers. GaAs has a bandgap of 1.43 eV, while InP features a bandgap of 1.35 eV, which make them efficient light emitters at specific wavelengths.
Innovations with Bandgap Engineering
Bandgap engineering involves the modification of the bandgap energies of semiconductors. This approach enables the creation of materials with tailored bandgaps for specific applications. For instance, altering the composition of AlGaAs, a compound semiconductor, can adjust the bandgap from 1.42 eV (GaAs) to 2.16 eV (AlAs).
Impact of Bandgap on Applications
The bandgap energy of a semiconductor determines its colour sensitivity for photodiodes, its breakdown voltage for power devices, and its light emission range for LEDs. For example, semiconductors with larger bandgaps can tolerate higher energies and are more suitable for high-temperature or high-voltage applications.
Conclusion
The properties of semiconductor materials are intimately tied to their bandgap energies. Understanding the relationship between the bandgap and the performance of various materials can guide the design of more efficient semiconductor devices. From silicon’s ubiquitous applications to the high-performance characteristics of wide bandgap semiconductors, the scope and potential of these materials continue to grow, shaping the future of the semiconductor market.
Comments are closed.